HEART FAILURE (HF): HOW THE LATEST SCIENCE SUPPORTS THE USE OF PULSED ELECTROMAGNETIC FIELDS (PEMFS) TO REVERSE OR SLOW THIS PROGRESSIVE AND LETHAL CONDITION
Table of Contents
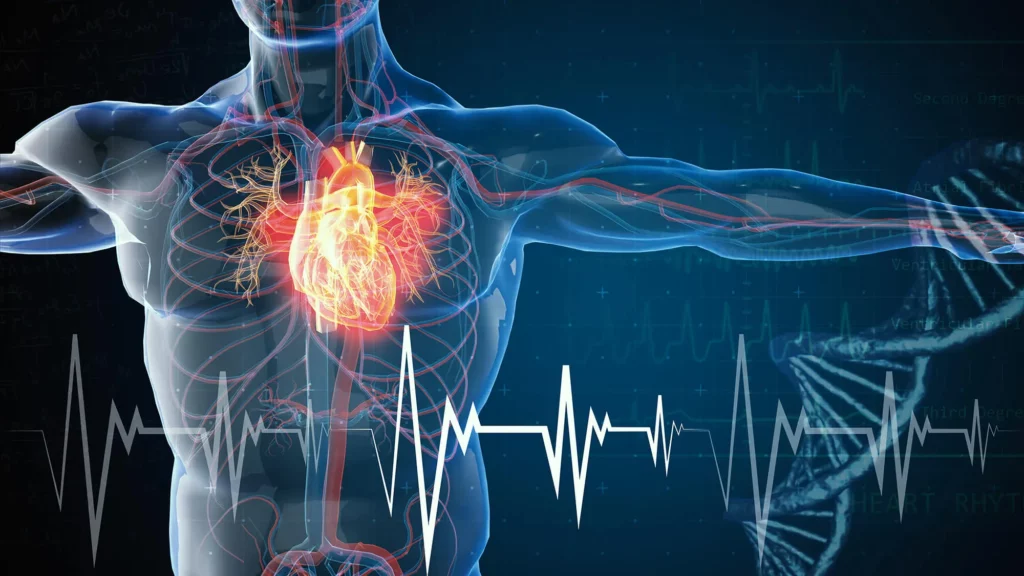
Heart failure (HF) affects 6.2 million people in the United States of America, with close to 500,000 new people diagnosed each year. 50% of those with HF are readmitted to the hospital within 6 months after treatment. In 2017, HF accounted for 80,480 deaths. 75% of those with HF have pre-existing hypertension. Given that HF is a progressive disorder, about 50% of those with HF will die within 5 years. Conventional medical treatments slow progression but are not a cure or reverse the condition. Nonconventional treatment approaches are clearly needed.
Research in the last 10 years has provided a better understanding of the underlying heart mechanisms involved in the development and progression of HF. This information reveals the opportunity for newer treatment approaches. When HF is associated with reduced output from the heart, that is, the ejection fraction, the processes that contribute to this are due to poor heart muscle contractions, general heart muscle health, increased sympathetic nervous system activity in the body, imbalanced neuroendocrine reactions, reduced nitric oxide sensitivity, ATP depletion, increased reactive oxygen species, cardiac scarring (fibrosis) and elevated heart cell death rate.
Some of these mechanisms include reduction of ATP production, poor intracellular calcium levels, dependence on glucose versus fatty metabolism, reduced cardiovascular supply, increased inflammation, aging of the vascular lining (endothelium) and fibrosis of the heart muscle. Pulsed electromagnetic fields (PEMFs) are known to safely impact all of these mechanisms and can be used to augment the benefits of conventional therapies.
Ideally, all individuals should be using PEMFs for preventive health maintenance throughout their lives, thus reducing the likelihood of development of heart failure through its various causes. However, once there is evidence of cardiac stress and heart tissue restructuring (remodeling), but before the diagnosis of HF is made, PEMFs are likely to have the most impact in reversing or slowing the progression of the subsequent, almost inevitable, development of HF.
The potential for PEMF therapy to help with heart failure (HF) was brought home to me about 20 years ago when I saw a woman who was previously very active and an avid golfer, confined to a wheelchair by her late-stage heart failure. Through a colleague, she received around 12 hours a day of therapy with a high intensity static magnetic therapy device over about two weeks. At the end of that treatment time, she was not only able to walk, but actually went out and played golf again. In the 30 years I had been a medical doctor at that time, I had never seen such dramatic recovery without the heavy-duty use of medications.
WHAT IS HEART FAILURE?
Heart failure syndrome is the inability of the heart to deliver adequate blood to the body to meet metabolic needs and oxygenation of the body at rest or during mild exercise. Heart muscle (myocardial) dysfunction can be systolic, diastolic or both; acute or chronic; compensated or uncompensated; or involve one or both ventricles.
Several mechanisms in the body are activated to counter heart failure, depending on how long the heart failure has been present. These include neuroendocrine changes involving the sympathetic nervous system, renin-angiotensin hormones, the kidneys and other alterations that try to restore both the output of the heart and tissue circulation. The left ventricle can’t eject an adequate volume of blood (ejection fraction). The right ventricle cannot fill adequately due to stiffness. Left ventricular heart failure is the dominant picture of Heart Failure Syndrome, but the right heart can develop isolated failure as well. When both ventricles fail that is usually an indicator of an end-stage clinical situation of the heart failure syndrome.
HF is a progressive disease with a major impact on quality of life. Except for situations where the HF may be caused by treatments that can reverse the condition, HF usually worsens with time. Although some people survive many years, progression has an annual mortality rate of 10%. Overall, 80% of men and 70% of women with heart failure under 65 years of age can be expected to die within 8 years of the diagnosis. The rate of mortality in those over 65 with HF is even higher and occurs sooner.
WHAT ARE THE CAUSES OF HF?
HF is largely a condition of older people. Less than 10% of the population between 60 – 79 has HF, whereas, of those over age 80, between 10.8 – 13.5% have it. It is estimated that the prevalence of HF will increase 46% from 2012 to 2030, resulting in >8 million people ≥18 years of age with HF in the United States.
The most common causes of HF are ischemic heart disease (blocked coronary arteries), hypertensive heart disease, cardiomyopathy, rheumatic heart disease and other causes. Hypertension is the cause in 60% of those with heart failure over age 50. The percentage of increased risk of death in those with HF with atrial fibrillation (A Fib) is 340%; with pulmonary hypertension, 210%; and poor kidney function with low creatinine clearance, 98%. HF from blocked coronary arteries has been shown to be associated with higher likelihood of death compared to HF from other cardiac diseases.
Starting therapy for HF may lead to some control of the clinical condition – the stability phase. Months to years following the stability phase, function may decline leading to multiple hospitalizations. Eventually the condition may not respond to treatment when relatively permanent physical changes in the heart ventricles set in. The longer it takes for treatment to be started from the onset of symptoms and the initial HF diagnosis, the worse the outcomes, with the cutoff being 18 months. So, there is urgency to start aggressive early management of the HF.
Once refractory, heart failure is medically managed by continuous adjustments of pharmaceuticals and may end up with the use of left ventricular assist devices (LVADs) and cardiac transplantation. Unfortunately, not everybody is a candidate for these invasive end-stage treatments. LVADs are generally considered a bridge to eventual heart transplantation.
WHAT ARE THE DIFFERENT TYPES OF HEART FAILURE
The most common terms used to describe heart failure include:
- Left-sided heart failure – the most common type.
- Right-sided heart failure. The right heart ventricle is responsible for pumping blood to your lungs to collect oxygen.
- Diastolic heart failure.
- Systolic heart failure.
The four stages of heart failure
A – pre-heart failure: Firstly, having a family history of heart failure or several predisposing cardiac conditions
B – asymptomatic or silent heart failure: Secondly, systolic left ventricular dysfunction with an ejection fraction* (EF) of <=40% –
C – signs or symptoms of heart failure: Thirdly, shortness of breath, fatigue, reduced exercise tolerance and swelling of the feet, ankles, lower legs, and/or abdomen
D – advanced symptoms that don’t get better with treatment: Lastly, the final stage of heart failure, NYHA class III-IV, symptoms on mild or minimal exertion or at rest
*Ejection fraction (EF) is a measurement, expressed as a percentage, of how much blood the left ventricle pumps out with each contraction. Normal range: 52–72%. Severely abnormal: <30%.
The New York Heart Association (NYHA) Classification provides a simple way of classifying the extent of heart failure and is different from the above stages A-D. It classifies those with HF into one of four categories based on their limitations during physical activity; the limitations/symptoms relate to normal breathing and varying degrees of shortness of breath and/or angina pain.
NYHA Classification – Stages of Heart Failure:
- Class I – No symptoms and no limitation in ordinary physical activity, e.g., shortness of breath when walking, climbing stairs etc.
- Class II – Mild symptoms (mild shortness of breath and/or angina) and slight limitation during ordinary activity.
- Class III – Marked limitation in activity due to symptoms, even during less-than-ordinary activity, e.g., walking short distances (20—100 m). Comfortable only at rest.
- Class IV – Severe limitations. Experiences symptoms even while at rest. Mostly bed bound individuals.
There is a further classification of heart failure based on the ability of the heart to pump out blood. This is called the ejection fraction (EF). The two forms of HF related to the EF are heart failure with preserved ejection fraction (HFpEF) and heart failure with reduced ejection fraction (HFrHF).
The terms congestive heart failure (CHF) and heart failure (HF) have been used synonymously. However, with CHF, the heart’s capacity to pump blood cannot keep up with the body’s needs. As the heart weakens, blood begins to back up and force liquid through the capillary walls. The term “congestive” refers to the resulting buildup of fluid in the ankles and feet, arms, lungs, and throughout other body organs, including the belly and the brain. CHF would normally be considered starting in stage C or NYHA class III. So, not all individuals with HF would be considered to have CHF.
WHAT HAPPENS IN THE HEART AND BODY BECAUSE OF HF?
Heart failure is caused by abnormal function of different parts of the heart including the lining around the heart (pericardium), the heart muscle itself (myocardium), heart muscle cells (cardiomyocytes), the lining of the chambers of the heart (endocardium), the heart valves and the blood vessels – large (macro) and small (micro). Problems with any of the parts of the heart individually can lead to HF.
In HF there is a lower amount blood pumped out of the heart (cardiac output) but not necessarily decreased EF. Symptoms are due to a decreased output of blood into the rest of the body (forward blood flow), with resulting backup into the lungs. The body tries to compensate for the low output by physiological responses that increase the amount of blood coming into the heart (preload) and going out into the rest of the body (afterload). Preload and afterload problems are in a vicious cycle. These compensation actions lead to worsening of heart malfunction and increased HF symptoms.
Reduced function of the heart muscle can result from decreased ability of the heart muscles to contract properly and/or due to stiffening of the muscle walls (fibrosis). This means that the heart chambers cannot get rid of all the blood inside them with every contraction and relaxation (reduced EF), resulting in the backup of blood in the body or the lungs. The pressure in the blood vessels from the backup leads to leakage of fluid into the lungs and throughout the body, hence, congestion. Commonly in HF the heart enlarges (cardiomegaly) as it attempts to function better in the face of the causes and consequences of the HF. This can be further affected by the proper flow of electrolytes in/out of heart muscle, essential to preserve cardiac function.
The endothelium is a very thin lining of metabolically active cells inside the blood vessel wall. A common cause of HF is impaired cardiac blood vessel endothelial function, which is related to, not only local, but also systemic microvascular inflammation. This is due to underlying coexisting conditions such as hypertension, obesity, ischemia, diabetes, metabolic syndrome, lung disease, smoking, and iron deficiency.
When HF is associated with a reduced ejection fraction (EF), the processes that contribute to this are due to poor muscle contractions, general heart muscle health, increased sympathetic activity in the body, neuroendocrine reactions, reduced nitric oxide sensitivity, ATP depletion, increased reactive oxygen species, and elevated heart cell death rate.
Autoimmune diseases may also contribute to systemic inflammation that leads to endothelial inflammation and dysfunction. By itself, nitric oxide leads to smooth muscle relaxation and decreased thickening of the blood vessel wall. ATP, of course, is necessary for muscles to beat and contract adequately and regularly. Also, cardiac muscle cells under stress are more prone to premature death (apoptosis).
So, the longer HF has been in place, the more rapid the decline. All the effects of HF are cumulative and increasingly irreversible as the heart remodels itself, despite optimized medical treatments. Those with a longer duration of HF are older and more likely to have multiple coexisting conditions (e.g., A Fib, renal dysfunction) or HF-related physiological changes. Longer HF duration is independently associated with more advanced irreversible myocardial damage. (Sugiura)
CONTRIBUTING FACTORS TO THE DEVELOPMENT AND PROGRESSION OF HF
Multiple factors, whether acting individually or synergistically, contribute to the development and progression of HF. These can include: reduced energy supply (ATP), tissue calcium imbalance, poor tissue metabolism, cardiac and peripheral vascular changes, inflammation, gastrointestinal blood supply, endothelial dysfunction, and scarring or fibrosis of cardiac tissue. Enlargement of the heart or cardiac hypertrophy is a key aspect of HF.
ATP (adenosine triphosphate) and the Heart
Every action in every cell, anywhere in the body, directly or indirectly, requires ATP. ATP is the fundamental “currency” supplying energy for
- muscle contractions
- ion movements
- production of both small molecules, such as ATP, and large molecules, such as the proteins, RNA, and DNA.
The majority (~95%) of the ATP used by the heart comes from the metabolism of oxygen in the mitochondria. Mitochondria are the “powerhouses” of the cell. Mitochondria inside each cell take up about 40% of the volume of heart muscle cells (cardiomyocytes). By contrast, in liver cells they only take up about 20-25% of their cell volume. Each ATP molecule is recycled 1000-1500 times per day. Therefore, the human body turns over its weight in ATP daily, or about 65 Kg/day in a resting adult. (Zimmerman) Only about 80-100 grams of ATP are stored in the body at one time. This is enough energy for 5-8 seconds of all-out effort.
During high-intensity exercise, the heart uses more than 90% of its maximal oxidative capacity, meaning there is minimal extra capacity over what is being used for the exercise. Oxygen consumption and cardiac work need a constant, readily available supply of ATP. (Ventura-Clapier) The bioenergetics of the heart is tightly controlled. In HF, there is an imbalance between the work the heart must do and the energy it makes to fulfill its needs. The failing heart has energy starvation.
The requirement for ATP is absolute.
The human heart contains ~0.7 grams of ATP. But, to maintain normal electrical action and continuous contractions, it uses ~6000 grams (6 Kg) of ATP a day! This is about 10% of the total body need for ATP per day. All ATP in a normal heart needs to be renewed every ≈ 20 seconds. If ATP production suddenly stopped in a healthy human heart, the stored ATP would only be able to keep the heart beating for a few seconds. So, the rate of ATP use must be continually matched with the rate of ATP production – on a beat-to-beat basis! The heart is considered a ‘metabolic omnivore’ and can use multiple sources to produce ATP. (Zhou)
Many metabolic pathways must be controlled and integrated to supply the ATP needed for energy in cell processes including ion transport, muscle contraction, nerve impulses, phosphorylation, and making the biochemicals needed for repair and survival. (Dunn) So, heart muscle cells need ATP to maintain normal heart rates, pump blood and support increased work demands, i.e., to recruit its contractile reserve.
Based on all the above, ATP plays a critical role when there is a lack of blood supply to the heart (ischemia) and in the development and maintenance of heart enlargement (hypertrophy) and subsequent HF. Ischemia and HF, in a vicious cycle, fail to integrate to maintain normal and constant levels of production of ATP.
Cardiac hypertrophy
Enlargement of the heart (cardiac hypertrophy) is an early indicator of the risk of developing HF. Cardiac hypertrophy is abnormal or pathologic heart tissue restructuring (remodeling). Pathologic remodeling increases energy demand because of unfavorable heart shape, increased neurohormonal stimulation, and impaired calcium function. (Zhou) Together, these changes upset the balance (homeostasis) between energy supply and demand, resulting in cardiac energy management stress, hence, an energy starved heart. One way to reduce energy demand on the heart is through standard medical therapy (such as vasodilators and beta blockers), which improve survival in heart failure. These treatments effectively reduce energy consumption by improving cardiac function. Nevertheless, conventional medical approaches do not increase the supply of ATP, hence the continuing, inevitable downward spiral of HF.
However, there is an ATP paradox in HF. The ATP content in a failing heart is largely maintained until the end stage, despite a mismatch between energy supply and demand. The stressed heart continues on a downward spiral to failure despite relatively stable ATP. The mitochondria play a role during cardiac remodeling to keep energy balance, but adequate ATP supply alone can’t stop the other non-energy-dependent processes in HF from leading to the vicious cycles of heart failure. (Zhou)
Intracellular calcium
Abnormal calcium ion (Ca2+) imbalance is a hallmark of HF. Ca2+ movement is impaired in failing hearts and results in reduced energy charge during cardiac electrical activity. Mitochondria act as a Ca2+ sink under pathological conditions, resulting in Ca2+ overload that contributes to mitochondrial dysfunction. Ca2+ is an important regulator of mitochondrial function and involved in the development of HF. But, uptake of Ca2+ into mitochondria is reduced in failing hearts. (Zhou) PEMFs appear to increase intracellular Ca2+ (Barbier), providing yet another possible mechanism of PEMFs aiding HF.
Fatty acid metabolism
Beat-to-beat cardiac ATP production happens by oxidative metabolism in mitochondria using fatty acids as the primary fuel. (Zhou) During pathological heart remodeling, cardiac metabolism shifts toward glucose; and fatty acid oxidation and fatty acid metabolism decrease. ATP generated from the use of glucose alone is less than 5% of the total ATP used in a normal adult heart. The capacity for glucose-derived ATP production is limited in adult hearts. The increased reliance on glucose reduces the efficiency of ATP production and makes pathological remodeling worse. Since fatty acid metabolism is not as efficient, lower fatty acid oxidation increases accumulation of more damaging incompletely oxidized fatty acids. This mismatch between fatty acid supply and oxidation is seen early in HF.
Vasculature and HF
There is much more awareness of the large coronary arteries (macrovascular circulation) that feed the heart muscle and get stented or bypassed when blocked. The macrovascular blood supply feeds the smaller, capillary blood vessels. It is the tiny capillary blood vessels (microvascular circulation) that do most of the work to supply circulation to the heart, down to the cellular level. The number of capillaries in a given area of tissue (capillary density) is critical for the supply of blood at the tissue level. Both lower capillary density and obstructed vascular flow in capillaries can impact cardiac muscle function.
Furthermore, heart failure (HF) patients can have body wide, macrovascular or microvascular, constriction and reduced peripheral blood supply. The benefits from improving blood flow in HF have been known for a long time. (Luxán) For example, nitroglycerine and other nitrates/nitrites, which release nitric oxide (NO), have been used in heart disease since 1847 (Lee) because of their vasodilating benefits. Angiotensin-converting enzyme inhibitor drugs, called ACE inhibitors, commonly used in treating HF, stimulate NO release. PEMFs have been found to increase the activity of ACE inhibitors. (Sadeghzadeh)
Moreover, chronically reduced blood supply can lead to chronically ischemic HF. Ischemic HF has reduced capillary density and inflamed endothelium. There is also significant chronic heart muscle cell (cardiomyocyte) death plus compensatory enlargement of the heart. As a result, surviving heart muscle cells have a compensatory increase in size followed by enlargement of the heart chambers, reduced numbers of capillaries and inflamed endothelium, scarring and increased numbers of inflammatory macrophage white blood cells. This is the classic situation in HF with preserved ejection fraction (HFpEF). So, HFpEF has scarring (interstitial fibrosis), sporadic cardiomyocyte death, and hypertrophy. On the other hand, HF with reduced EF (HFrEF) is associated with impaired coronary flow reserve and microvascular perfusion.
Macrovascular narrowing or occlusion of the coronary arteries leads to reduced blood supply to the myocardium. This leads to secondary microvascular problems. But microvascular dysfunction can occur in the absence of coronary artery occlusion. Metabolic syndromes and diabetes, as well as hypertension, affect the coronary microcirculation. Maladaptive hypertrophy is also associated with reduced vessel density. Fewer capillaries lead to decreased oxygen supply to the enlarged heart muscle.
Blood vessels of the heart not only regulate local blood flow but also control cardiac cell metabolism. The metabolic requirements of the heart to fulfill its pumping function are immense. The vasculature is essential for regulating cardiac metabolism and protecting against HF.
In HfrEF from coronary artery disease, there is also an increase in reactive oxygen species (ROS), reduction of NO and inflammatory activation in the microvasculature. These then lead to reduced microvascular circulation and endothelial dysfunction. The endothelium then may start shedding itself. Restoring endothelial function is expected to restore vascular flow and oxygen supply and rescue dysfunctional cardiomyocytes.
Recovering microvascular function is especially important for those who have a stunned or hibernating myocardium. A stunned myocardium results when the coronary blood supply is improved after prolonged postischemic dysfunction, such as in heart ischemia episodes or myocardial infarction. Hibernating myocardium is chronically ischemic myocardium supplied by a narrowed coronary artery in which ischemic cells haven’t died but contraction is chronically depressed.
Preserved EF HF (HFpEF) is becoming the predominant form of HF in aging societies. There is an important link between coronary microcirculatory dysfunction and HFpEF. HFpEF involves all the muscular structures of the heart. Cardiac microvascular endothelial cells regulate the relaxation of cardiomyocytes, especially through NO activity. Heart muscle cells don’t relax properly when endothelial cells are inflamed. This leads to increased stiffness (fibrosis) of the left ventricle with diastolic dysfunction. There is some evidence that PEMFs induce NO in various circumstances, contributing to increased microvascular blood flow and secondarily to reduced development of hypertrophy. (McKay)
Inflammation in HF
It has long been known that heart failure (HF) is associated with measures of systemic inflammation. (Murphy) However, even though the association between inflammation and HF severity and prognosis is well known, clinical trials of conventional anti-inflammatory therapies have not been successful. Targeted anti-inflammatory therapies with non-conventional approaches may be better to improve prognosis in HF. Inflammation in the heart is seen in all HF, but there is more inflammation in those with HFpEF. This is because HFpEF is more associated with diabetes, hypertension, chronic obstructive pulmonary disease, obesity, and chronic kidney disease, all conditions with high levels of inflammation. So, the therapeutic target in HFpEF, should be the reduction of systemic inflammation, not just cardiac inflammation.
57% of HF patients enrolled in one study (Redfield) had elevated serum C-reactive protein (CRP), a common measure of systemic inflammation. Those in stable chronic HF with reduced and preserved EF had a median elevated high sensitivity CRP (hsCRP) between 6.6 mg/l and 8.5 mg/l, with optimal levels being less than 1. (Watanabe) Systemic inflammation is even greater in acute HF, with hsCRP concentrations of 12.6 mg/l.
Other markers of inflammation are also elevated in HF. These include tumor necrosis factor (TNF)-a, IL-1b, IL-6, and galectin-3. TNF-a is associated with both impaired systolic and diastolic function, and adverse cardiac remodeling. Any cardiac cell injury leads to increased interleukin (IL)-1. IL-1b reduces energy production and myocardial contractility through direct effects on mitochondria. IL-1 also impairs diastolic function by affecting intracellular calcium reuptake which inhibits cardiomyocyte relaxation. Nuclear factor kappa B (NFkB) is decreased. It is needed to reduce inflammation, cell death (apoptosis), extracellular matrix remodeling, mitochondrial dysfunction and induces antioxidant effects.
Targeting these inflammatory factors is important in managing HF. Anti-IL-1 therapy has been evaluated in those with recently decompensated HF (Murphy). The death or hospitalization rate for HF after therapy was lower with longer-term therapy. Anti-inflammatory therapies may take longer to have benefit than the shorter acting benefit seen with conventional medical therapies with heart action-altering mechanisms.
Reducing IL-12 and IL-23 can also be helpful. IL-12 may induce autoimmune myocarditis and microvascular endothelial dysfunction. IL-23 increases myocardial remodeling and decreases survival post–myocardial infarction (MI) in animals. Reducing IL-6 and the important N-terminal pro–B-type natriuretic peptide (NT-proBNP) also help. (See more on ProBNP below).
Inflammation and body fat. Another important target in reducing inflammation in the body is to address excess body fat, which increases the risk for HF. (Harada) Adipose tissue, especially belly fat, secretes a variety of cytokines, also referred to as adipokines, which have a higher likelihood of pro-inflammatory actions. Conversely, the production of adiponectin, an anti-inflammatory adipokine that inhibits cardiac hypertrophy, inflammation, and fibrosis, is suppressed in obesity.
The cardiac fat pad adds additional risk for HF. There can also be significant fat accumulation around the heart. In addition to the increased cardiovascular risk from visceral obesity, it has recently been found that increased fat around the heart, called epicardial adipose tissue or fat (EAT) creates an additional risk for the heart. (Mookadam) It is also often called the pericardial fat pad. This is usually detected on chest x-rays, chest CT scans or echocardiography. There is a correlation between the amount of visceral obesity and the EAT. The risk factors contributing to visceral obesity also apply to EAT.
EAT increases the mechanical load on the heart, releases adipokines and cytokines, metabolically modulates adjacent cardiac tissue, diffuses free fatty acids directly into adjacent heart cells, and contributes to the overall metabolic burden of obesity in the body. EAT > 5 mm is associated with left atrial enlargement, lower ejection fraction, increased size of the left ventricle and abnormal diastolic function. All of these can contribute to heart failure.
Innate immune system. Activation of the innate immune system contributes to the inflammatory milieu in HF. (Murphy) The toll-like receptor four (TLR4), which has the highest amount in the heart, contributes to the myocardial inflammation that occurs in HF, myocarditis, ischemia-reperfusion injury, aortic valve disease, hypertension and atherosclerosis. TLR4 expression is increased in patients with advanced HF. Inhibiting TLR-4 has been found to reduce IL-1b and IL-6 concentrations and lessen cardiomyocyte hypertrophy in response to pressure overload in animal models of HF.
70% of patients with end-stage HF have anti-cardiac antibodies, which may be directed against a variety of cardiac proteins or enzymes. (Murphy) Although only <1% of the healthy population have autoantibodies against b1-adrenergic receptors, up to 60% of patients with nonischemic cardiomyopathy and >90% of LV assist device recipients have them. Removing these antibodies has been shown to improve cardiac function and left ventricular EF.
Skeletal muscle effects of inflammation. Beyond the negative effects these antibodies have on the heart itself, inflammatory cytokines also affect skeletal muscle oxygen extraction during exercise, worsen anemia and loss of muscle mass, promote sodium retention in the kidneys leading to plasma volume expansion, and increase pulmonary pressures during exercise due to pulmonary vasoconstriction. All of these contribute to shortness of breath and reduced exercise tolerance. Yet another reason that dealing with whole body inflammation reduction is needed for a more comprehensive approach to controlling heart failure.
Sterile inflammation. The hemodynamic stress of HF induces a state of sterile inflammation, whereby increased heart muscle wall tension and mechanical stretch trigger the release of an array of proinflammatory cytokines by cardiomyocytes and cardiac fibroblasts, including TNF-a, IL-6, IL-1b, angiotensin II, and myostatin. (Murphy) HF itself also triggers mitochondrial dysfunction, generates reactive oxygen species, and leads to activation of the NLRP3 inflammasome, with subsequent maturation of proinflammatory cytokines, such as IL-1b and IL-18. Simultaneous with the production of inflammatory cytokines from within the heart, inflammation occurs as a result of activation of the innate immune system, neurohormonal activation, and oxidative stress, and through cross-talk with other organ systems.
Ischemia of the gut mucosa.
Later stages of HF may lead to ischemia of the intestinal mucosa by either a decrease in cardiac output or venous congestion in the setting of right-sided HF and elevated venous pressures. Gut mucosal ischemia alters its permeability and the consequent impaired barrier function allows crossing over of endotoxins, microbial components, and metabolites into the systemic circulation. Released endotoxins decrease after successful treatment of decompensation or following diuretics. HF is also associated with dysbiosis of the gut microbiome, with low bacterial diversity and depletion of butyrate-producing bacteria. Butyrate exerts anti-inflammatory effects and stimulates regulatory T cells, which have a key role in limiting the inflammatory response.
Endothelial senescence
Senescence is a protective response from organisms against stress that limits the proliferation of aged non-functional cells. However, senescent cells accumulate in fibrotic regions and there is cumulative evidence that senescence is closely related to cardiovascular disease. (Gevaert) Indeed, endothelial cell senescence is associated with an augmented dysfunction and vascular inflammation. Recent studies further demonstrated that endothelial senescence contributes to HFpEF.
Fibrosis and HF
Fibrosis, also known as fibrotic scarring, is a form of wound healing in which connective tissue replaces normal tissue. To the extent that it goes unchecked, it can lead to significant tissue remodeling and the formation of permanent scar tissue.
A key mechanism of HF is cardiac remodeling, which includes two aspects: cardiomyocyte injury and myocardial fibrosis. (Liu) Cardiomyocyte injury presents as cardiomyocyte hypertrophy, necrosis, and apoptosis. Repeated cellular injuries, chronic inflammation and repair are susceptible to fibrosis where excessive accumulation of extracellular matrix components, such as the collagen is produced by fibroblasts, leading to the formation of a permanent fibrotic scarring and thickening of the affected tissue. Fibrosis acts to deposit connective tissue, which can interfere with or totally inhibit the normal structure and function of the organ or tissue. Mast cell deposition in heart muscle leads to fibrotic and inflammatory reactions. Mast cell density is increased in ischemic cardiomyopathy and hypertension.
There are two types of heart fibrosis: replacement and interstitial fibrosis. Replacement fibrosis usually occurs after heart cell necrosis from myocardial infarction. It can also occur from hypertrophic cardiomyopathy, sarcoidosis, myocarditis, chronic renal insufficiency, and toxic cardiomyopathies. Interstitial fibrosis is diffuse, and includes reactive and infiltrative interstitial fibrosis. Reactive fibrosis is seen in many diseases, including hypertension, and in aging. Infiltrative fibrosis is less common and caused by the progressive deposition of proteins (amyloidosis) or glycosphingolipids in the space between cells. Eventually, both interstitial and infiltrative fibrosis can lead to heart cell apoptosis and replacement fibrosis.
Assessment of the degree of fibrosis can be evaluated using blood markers or cardiac magnetic resonance imaging (CMR). There are two myocardial fibrosis blood markers, galectin-3 and soluble ST2, which can be used for risk stratification and detection of risk even without heart failure. CMR imaging can effectively detect and evaluate the degree of myocardial fibrosis, edema and infiltrates in the heart, with various heart problems and especially in HF. (Liang)
N-terminal pro–B-type natriuretic peptide (NT-pro N-terminal BNP)
NT-proBNP is commonly used to monitor HF. Plasma BNP concentration increases with the severity of HF. If BNP < 100 pg/mL, HF is unlikely; BNP between 100 – 500 pg/mL, HF is more likely; with BNP >500 pg/mL, HF or cardiac dysfunction is probable and rapid therapy for HF is suggested. (Cao) HF is more likely in those less than 50 years old with NT-proBNP levels > 450 pg/mL; between 50 and 75 years old with NT-proBNP levels > 900 pg/mL; and if more than 75 years old with NT-proBNP levels > 1800 pg/mL
The reduction of elevated BNP and NT-proBNP levels in HF predicts an improvement in clinical symptoms. A study of acute myocardial function patients found that BNP and NT-proBNP predicted sudden cardiac death and were the strongest predictors, even after adjusting for clinical variables, including EF. Plasma BNP and NT-proBNP can be used clinically to guide the management of individuals with HF and cardiac dysfunction, and they are also used as prognostic indicators which can help clinicians adjust their therapy strategy. BNP and NT-proBNP are still underused by clinicians in the management of HF.
Common medical treatments for HF
The conventional medical treatments for HF are based on the stage of HF.
Type of Heart Failure (HF) | Conventional Therapies |
Acute decompensated HF | oxygen, water pills (diuretics), vasodilator pills, ACE inhibitors and/or muscle contraction enhancers |
Chronic HF | hypertension control, water pills, ACE inhibitors, ARBs, beta blockers, aldosterone antagonists, etc. Beta-blockers, ACE inhibitors, and aldosterone antagonists are called the “Golden Triangle” of HF treatment |
Advanced HF | persistent or progressive severe symptomatic state with decreased cardiac function despite guideline-based optimized medical and device therapy – consideration given to advanced cardiac surgery options, such as heart transplant or left ventricular assist device (LVAD) implantation, or to initiate the management of end-of-life care |
Expanded therapeutic strategies
While the above common, conventional medical treatments for HF focus on the medical aspects of HF, strategies that target the many other aspects of the pathophysiology of the condition are rarely considered. Adding several interventions to target these known pathophysiological aspects should be able to improve function and extend the life of those suffering with HF.
It has been shown that in HF there are: poor heart muscle contractions, reduced general heart muscle health, increased sympathetic activity in the body and related neuroendocrine reactions, reduced nitric oxide levels and sensitivity, ATP depletion, increased reactive oxygen species, elevated heart cell death rate (apoptosis) and an increased rate of autoimmune diseases.
The known pathophysiological aspects that should be addressed with multiple coordinated strategies include the following:
- enhancing cardiomyocyte relaxation, endothelial metabolism, medication effectiveness, nutrient transport, rehabilitation.
- improving afterload reduction and autophagy.
- increasing protection from atherogenesis, ATP production, circulation, GAG production and oxygenation.
- reducing abnormal angiogenesis and collateral formation, growth factors, infection and inflammation.
- stimulating muscle and vascular repair.
When there is atherosclerotic plaque significantly obstructing coronary blood vessel flow, the heart will attempt increasing angiogenesis and collateral formation. These can be important and valuable self-preservation strategies initiated by the heart. However, these reactions are a double-edged sword by virtue of enhancing the development of hypertrophy. By reducing several of the other pathophysiologic aspects of HF, one may decrease the need of the heart tissue to make these vascular changes.
As yet there are no proven effective and safe anti-fibrosis medications available. Herbal therapies may have some effectiveness in decreasing cardiac fibrosis. (Li X; Wang J) At the moment the best strategies are preventive, other than the probable effectiveness of PEMFs, discussed below.
ROLES OF PEMF FOR HF
Inflammation
A very important aspect of controlling inflammation is by stimulating a ubiquitous molecule in the body called adenosine, acting through its generalized receptor, the adenosine receptor (AR). Adenosine regulates the function of every tissue and organ in the body and is considered a “guardian angel” in human disease (Borea). Release of adenoisine is enhanced with PEMF stimulation, inflammation, pH change, hypoxia, tissue damage, or nerve injury in all the tissues of the body. (Varani) The concentrations of adenosine are naturally at physiologically low levels in body fluids between the cells of unstressed tissues. These concentrations increase rapidly in response to cell injury-causing stress conditions such as low oxygen (hypoxia), lack of blood supply (ischemia), inflammation, or trauma.
PEMFs stimulate the activation of adenosine receptors (ARs), increase their functionality, and augment chemical agents that also stimulate these receptors. PEMFs primarily influence A2A and A3 ARs. A2A AR is the predominant receptor subtype responsible for coronary blood flow regulation. Stimulation of the A2A AR dilates coronary arteries in both an endothelial-dependent and -independent manner. (Mustafa) Stimulation of A2A and A3 ARs by PEMFs in cells throughout the body results in reduction of inflammation by lowering many proinflammatory tissue cytokines, including reduction of: tumor necrosis factor-α (TNF-α), Interleukins IL-1β, IL-6, and IL-8 and NF-kappa B.
Immunomodulatory
PEMF therapy has immunomodulatory effects to decrease the production of proinflammatory cytokine production, while stabilizing or increasing anti-inflammatory cytokine production and NF-kB expression during inflammation activation. PEMFs help to restore inflammatory cascades to homeostatic (healthy) production levels. (Ross)
Obesity and body fat
PEMFs have also been shown to reduce inflammation associated with obesity. (Baranowska; Du) Since the visceral/abdominal fat compartment is the greatest source of inflammation with obesity, applying PEMFs to a large part of the abdomen with a sufficient intensity daily is expected to help significantly. This would be true for any level of obesity, for existing inflammation and for preventing the damaging consequences of the secondary inflammation caused by abdominal cytokines released to circulate throughout the body. A better option would be to apply sufficient intensity whole-body PEMFs to help not only visceral, abdominal, fat and the fat under the skin but also the systemic effects of the inflammation caused by any excess fat. Generally, a fairly high intensity (equal to or greater than about 2000 gauss (200 mT)), whole body PEMF system would work best to deal with the broadest area of inflammation throughout the body.
Multiple physiologic actions
PEMFs have been shown to have numerous physiologic actions that address many of these known pathophysiologic aspects of HF (see Pawluk, Section 2: How PEMFs affect the body). As a result, PEMFs may be ideally suited for sole therapy and/or to complement other approaches in the treatment of HF. This would be especially true when working to control cardiac hypertrophic remodeling. PEMF therapy should be started early in the course of development of HF, and especially when cardiac hypertrophy is already seen, even before HF is diagnosed. The ideal time to begin daily PEMF treatment would be when the EF is already seen to be decreasing, before symptoms or signs of HF remodeling become evident. Once HF has already been diagnosed and medications have been begun, PEMF therapy should make HF medications even more effective and may allow the reduction of dosages, thus limiting side effects. (Pawluk)
ATP and mitochondrial function
There is a significant need to increase ATP production and improve mitochondrial function, and PEMFs are the ideal tool to do so. It has been shown that using PEMFs for only 20 minutes can stimulate ATP production (Zhang S) up to 600%, averaging 111-241%. However, since ATP is constantly recycled, frequent PEMF treatment to the heart may be needed to maintain ATP production and utilization. At this point it is unknown how long and how much of the ATP produced by PEMF stimulation will last. If a significant benefit is seen in cardiovascular function with PEMF therapy, attention should be paid to how long that benefit lasts and how often PEMF therapy should be repeated. It’s possible that with higher intensity PEMFs, treatment times could possibly be reduced to 10 – 15 minutes, multiple times a day depending on response.
Stem cells
The heart is continually making stem cells both for health maintenance and to replace natural cell turnover and for repair, especially in the presence of ischemia. PEMFs have been found to increase neural stem cells (Goodwin) as well as many other types of stem cells in the body (Maziarz; Poh). Research has also found that extremely low-frequency PEMFs tuned by ion cyclotron resonance for the calcium ion (Ca2+-ICR) could be used to drive cardiac-specific differentiation in adult cardiac progenitor cells without any pharmacological or genetic manipulation of the cells, increasing the lifespan of cardiomyocytes. (Gaetani)
Angiogenesis
Disruption of the natural coordinated tissue growth and angiogenesis in the heart contributes to the progression from adaptive cardiac hypertrophy to heart failure. Supplementation of angiogenic factors during progression from adaptive to maladaptive cardiac hypertrophy preserves cardiac function. Cardiac microvascular endothelial cells (CMECs) increase myocardial angiogenesis. Cardiac myocytes (CMs) also play a crucial role in myocardial angiogenesis. Pulse-burst magnetic field stimulation could augment angiogenesis, with associated improvement in ventricular function and reduced infarct size as found when studied in vitro in rats.
(Li F) The peak magnetic field of the coils used was 18mT. The PEMF promoted the proliferation and migration of CMECs and cardiac myocytes (CMs) directly. The PEMF also had an effect on the intercellular communication between CMECs and CMs. These results show novel cardiac cell mechanisms for PEMF action, indicating potential application in the treatment of ischemic myocardial disease and pathological cardiac hypertrophy. Thus, the use of PEMFs to promote angiogenesis in hypertrophic myocardium becomes a new therapeutic target of heart failure. Therefore, PEMF may potentially improve pathological myocardial hypertrophy and ischemic myocardial disease.
Cardiac fibrosis and myocardial stiffness
As far as the development of cardiac fibrosis is concerned, PEMFs have been shown to decrease the development of fibrosis in orthopedics. (Huegel) PEMFs have not been studied directly on cardiac fibrosis. However, PEMFs can indirectly affect the development of cardiac fibrosis by decreasing the cardiac inflammation that leads to fibrosis.
Arrhythmias and atrial fibrillation
Firstly, Arrhythmias and atrial fibrillation are common in those with heart failure, as a cause or consequence. PEMF therapy may be helpful in controlling or reducing the frequency and severity of these arrhythmias. Low-frequency, high intensity PEMF (LF-hiPEMF) could suppress atrial fibrillation by mediating the heart’s own natural autonomic nervous system. Left stellate ganglion (LSG) – located just above the first rib in the lower neck – autonomic neural activity can affect ventricular arrhythmia.
Studies have shown that LSG hyperactivity appears to predispose to the development of ventricular arrhythmia. The effect of LF-hiPEMF stimulation of has been studied in acute heart attack research. (Wang S) LF-hiPEMF stimulation reduced both the neural activity of the LSG and the incidence of ventricular arrhythmia. Therefore, this form of PEMF stimulation could be a novel noninvasive substitute for the existing implant device-based electrical stimulation or sympathectomy approaches, conventionally used in the treatment of arrhythmias.
Moreover, other research has also shown that lower intensity PEMFs applied directly to the heart in a dog model decreases the risk of episodes of atrial-fibrillation (Scherlag). In addition, see: Atrial Fibrillation (A-Fib).
Functional capacity limitation in HF
Additionally, generalized functional capacity in HF can be severely limited and debilitating. The functional capacity limitation in heart failure can be due to many factors not directly related to heart muscle function. Consequentially, rather, the cause may be the result of peripheral muscle changes. (Sbruzzi) These peripheral changes include decreased blood supply in the periphery, reduced capillary function, transformation of slow-twitch type I to fast-twitch type II fibers and changes in general metabolic and nutritional status that include reduced skeletal muscle size (atrophy/sarcopenia) and muscle strength. These changes are predictors of both exercise intolerance and the poor prognosis of the value of muscle training. If able to be accomplished, functionally useful muscle training has the potential benefit of increased maximum oxygen consumption, muscle mass (type I fibers), oxidative enzyme levels, endothelial function improvement and better performance in functional tests.
Moreover, Neuromuscular electrical stimulation improves peak O2, walking distance, quality of life, muscle strength, endothelial function, and depressive symptoms in patients with HF and could be important in cardiac rehabilitation for HF patients. (Neto) However, neuromuscular electrical stimulation (NMES), which is most commonly used, can be painful very uncomfortable. To provide adequate results, higher intensity levels of NMES are needed and therefore are of limited value. When NMES is used to treat weakened or atrophied muscles, high intensity stimulation is needed to generate sufficient deep muscle contractions. One study (Sbruzzi) found that NMES in individuals with HF produced only about one quarter of the peak intensity muscle contraction compared to maximum voluntary muscle contraction. While this may be useful in individuals with very weak local muscles and the inability to contract these muscles voluntarily, it would not be sufficient for general, whole-body rehabilitation.
On the other hand, neuromuscular magnetic stimulation (NMMS) is much more tolerable, especially for larger, deeper muscular enhancement. NMMS was studied in 40 healthy volunteers, 20 receiving active NMMS and 20 controls. (Yang) The active group received 15 minutes of quadriceps NMMS at maximum tolerable intensity three times per week for five weeks. The maximum magnetic field output intensity was 3.1 T/s. Visible muscle contractions were seen in all participants.
Furthermore, the tolerated NMMS intensity was about 45% of maximum possible. Even so, the isometric maximum and average peak torque of the NMMS group increased significantly by 22% and 23% respectively. The speed of being able to straighten the knee after stimulation also improved between 20 – 27%. The control group had no changes in their peak torques. This research indicates that the high intensity PEMF indicated for the stimulation of the heart in those with heart failure, would also be useful in peripheral muscular rehabilitation.
Conclusion
Finally, continued research into the pathophysiologic mechanisms of heart failure have discovered a number of changes that may be amenable to innovative therapies, such as PEMFs. Some of these cardiac changes include poor heart muscle contractions, reduced general heart muscle health, increased sympathetic activity in the body and related neuroendocrine reactions, reduced nitric oxide levels and sensitivity, ATP depletion, increased reactive oxygen species, elevated heart cell death rate (apoptosis) and an increased rate of autoimmune diseases. Root causes for some of these changes, whether acting individually or synergistically, include: Inflammation, fibrosis, cardiac hypertrophy, endothelial dysfunction, reduced energy supply (ATP), tissue calcium imbalance, poor tissue metabolism, cardiac vascular changes, metabolic tissue stress, dysregulated intestinal function, muscle weakness, hypoxia, and so on.
Lastly, as it happens, an innovative therapeutic strategy that addresses many of these dysfunctions, both as a cause and a consequence of HF is the use of pulsed elect magnetic field (PEMF) therapy. Evidence is accumulating for the value of PEMF therapy to address many of the pathophysiologic changes. Fortunately, there is increasing availability of PEMF devices. Research is desperately needed to determine the value of PEMF therapy as a complementary modality specific to the treatment of heart failure.
References
- Baranowska A, Skowron B, Gil K, Kaszuba-Zwoińska J. Obesity related adipokines release in rat adipose derived stem cell cultures influenced by pulsed electromagnetic field. Folia Med Cracov. 2018;58(2):131–145.
- Barbier E, Dufy B, Veyret B. Stimulation of Ca2+ influx in rat pituitary cells under exposure to a 50 Hz magnetic field. Bioelectromagnetics. 1996;17(4):303-11.
- Cao Z, Jia Y, Zhu B. BNP and NT-proBNP as Diagnostic Biomarkers for Cardiac Dysfunction in Both Clinical and Forensic Medicine. Int J Mol Sci. 2019 Apr 12;20(8):1820.
- Du L, Fan H, Miao H, Zhao G, Hou Y. Extremely low frequency magnetic fields inhibit adipogenesis of human mesenchymal stem cells. Bioelectromagnetics. 2014 Oct;35(7):519–30.
- Dunn J, Grider MH. Physiology, Adenosine Triphosphate. 2021 Feb 27. In: StatPearls (Internet). Treasure Island (FL): StatPearls Publishing; 2021 Jan–.
- Gaetani R, Ledda M, Barile L, Chimenti I, De Carlo F, Forte E, Ionta V, Giuliani L, D’Emilia E, Frati G, Miraldi F, Pozzi D, Messina E, Grimaldi S, Giacomello A, Lisi A. Differentiation of human adult cardiac stem cells exposed to extremely low-frequency electromagnetic fields. Cardiovasc Res. 2009 Jun 1;82(3):411-20.
- Gevaert AB, Shakeri H, Leloup AJ, et al. Endothelial Senescence Contributes to Heart Failure With Preserved Ejection Fraction in an Aging Mouse Model. Circ Heart Fail. 2017 Jun;10(6):e003806.
- Gomes Neto M, Oliveira FA, Reis HF, et al. Effects of Neuromuscular Electrical Stimulation on Physiologic and Functional Measurements in Patients With Heart Failure: a systematic review with meta-analysis. J Cardiopulm Rehabil Prev. 2016 May-Jun;36(3):157-66.
- Goodwin T and Dennis R. Physiological and molecular genetic effects of time-varying electromagnetic fields on human neuronal cells. NASA Johnson Space Center, Houston, TX, United States. NASA/TP-2003-212054.
- Harada T, Obokata M. Obesity-Related Heart Failure with Preserved Ejection Fraction: Pathophysiology, Diagnosis, and Potential Therapies. Heart Fail Clin. 2020 Jul;16(3):357-368.
- Huegel J, Chan PYW, Weiss SN, Nuss CA, Raja H, Waldorff EI, Zhang N, Ryaby JT, Soslowsky LJ, Kuntz AF. Pulsed electromagnetic field therapy alters early healing in a rat model of rotator cuff injury and repair: Potential mechanisms. J Orthop Res. 2021 Oct 17.
- Lee PM, Gerriets V. Nitrates. In: StatPearls (Internet). Treasure Island (FL): StatPearls Publishing; 2021 Jan-. Available from: https://www.ncbi.nlm.nih.gov/books/NBK545149/
- Li F, Yuan Y, Guo Y, et al. Pulsed magnetic field accelerate proliferation and migration of cardiac microvascular endothelial cells. Bioelectromagnetics. 2015 Jan;36(1):1-9.
- Li X, Li L, Lei W, Chua HZ, Li Z, Huang X, Wang Q, Li N, Zhang H. Traditional Chinese medicine as a therapeutic option for cardiac fibrosis: Pharmacology and mechanisms. Biomed Pharmacother. 2021 Oct;142:111979.
- Liang K, Baritussio A, Palazzuoli A, et al. Cardiovascular Magnetic Resonance of Myocardial Fibrosis, Edema, and Infiltrates in Heart Failure. Heart Fail Clin. 2021 Jan;17(1):77-84.
- Liu T, Song D, Dong J, et al. Current Understanding of the Pathophysiology of Myocardial Fibrosis and Its Quantitative Assessment in Heart Failure. Front Physiol. 2017;8:238. Published 2017 Apr 24.
- Luxán G, Dimmeler S. The vasculature: a therapeutic target in heart failure? Cardiovasc Res. 2021 Feb 23:cvab047.
- Maziarz A, Kocan B, Bester M, Budzik S, Cholewa M, Ochiya T, Banas A. How electromagnetic fields can influence adult stem cells: positive and negative impacts. Stem Cell Res Ther. 2016 Apr 18;7(1):54.
- McKay JC, Prato FS, Thomas AW. A literature review: the effects of magnetic field exposure on blood flow and blood vessels in the microvasculature. Bioelectromagnetics. 2007 Feb;28(2):81-98.
- Mookadam F, Goel R, Alharthi MS, et al. cross-sectional observational study. Heart Views. 2010;11(3):103-108.
- Murphy SP, Kakkar R, McCarthy CP, Januzzi JL Jr. Inflammation in Heart Failure: JACC State-of-the-Art Review. J Am Coll Cardiol. 2020 Mar 24;75(11):1324-1340.
- Mustafa SJ, Morrison RR, Teng B, Pelleg A. Adenosine receptors and the heart: role in regulation of coronary blood flow and cardiac electrophysiology. Handb Exp Pharmacol. 2009;(193):161-188.
- Mustafa SJ, Morrison RR, Teng B, Pelleg A. Adenosine receptors and the heart: role in regulation of coronary blood flow and cardiac electrophysiology. Handb Exp Pharmacol. 2009;(193):161-188.
- Pawluk W, Layne CJ. Power Tools for Health: how magnetic fields PEMFs help you. Publ. Friesen Press. 2017.
- Poh PSP, Seeliger C, Unger M, et al. Osteogenic Effect and Cell Signaling Activation of Extremely Low-Frequency Pulsed Electromagnetic Fields in Adipose-Derived Mesenchymal Stromal Cells. Stem Cells Int. 2018 Jul 12;2018:5402853.
- Redfield MM, Chen HH, Borlaug BA, et al. Effect of phosphodiesterase-5 inhibition on exercise capacity and clinical status in heart failure with preserved ejection fraction: a randomized clinical trial. JAMA 2013;309:1268–77.
- Ross CL, Zhou Y, McCall CE, Soker S, Criswell TL. The Use of Pulsed Electromagnetic Field to Modulate Inflammation and Improve Tissue Regeneration: A Review. Bioelectricity. 2019 Dec 1;1(4):247-259.
- Sadeghzadeh F, Entezari AA, et al. Characterizing the Binding of Angiotensin Converting Enzyme I Inhibitory Peptide to Human Hemoglobin: Influence of Electromagnetic Fields. Protein Pept Lett. 2020;27(10):1007-1021.
- Sbruzzi G, Schaan BD, Pimentel GL, et al. Effects of low frequency functional electrical stimulation with 15 and 50 Hz on muscle strength in heart failure patients. Disabil Rehabil. 2011;33(6):486-93.
- Scherlag BJ, Yamanashi WS, Hou Y, et al. Magnetism and cardiac arrhythmias. Cardiol Rev. 2004 Mar-Apr;12(2):85-96.
- Sugiura A, Kitahara H, Iwahana T, et al. Association of heart failure duration with clinical prognosis in advanced heart failure. Clin Res Cardiol. 2020 Mar;109(3):350-357.
- Varani K, Vincenzi F, Ravani A, et al. Adenosine receptors as a biological pathway for the anti-inflammatory and beneficial effects of low frequency low energy pulsed electromagnetic fields. Mediators Inflamm (2017) 2017:2740963.
- Ventura-Clapier R, Garnier A, Veksler V, Joubert F. Bioenergetics of the failing heart. Biochim Biophys Acta. 2011 Jul;1813(7):1360-72.
- Wang J, Li F, Zhou X, et al. Clinical observation of modified zhenwutang in treatment of chronic heart failure patients with yang deficiency syndrome, Chin. J. Exp. Tradit. Med. Formula (2018) 3–8.
- Wang S, Zhou X, Huang B, et al. Noninvasive low-frequency electromagnetic stimulation of the left stellate ganglion reduces myocardial infarction-induced ventricular arrhythmia. Sci Rep. 2016 Jul 29;6:30783.
- Watanabe E, Arakawa T, Uchiyama T, et al. High-sensitivity C-reactive protein is predictive of successful cardioversion for atrial fibrillation and maintenance of sinus rhythm after conversion. Int J Cardiol 2006;108:346–53.
- Yang SS, Jee S, Hwang SL, Sohn MK. Strengthening of Quadriceps by Neuromuscular Magnetic Stimulation in Healthy Subjects. PM R. 2017 Aug;9(8):767-773. Ts
- Zhang S, Clark M, Liu X, et al. The Effects of Bio-inspired Electromagnetic Fields on Healthy Enhancement with Case Studies. Emerging Science Journal 2019 Dec;3(6):369-381.
- Zhang Y, Li L, Liu X, et al. Examination of the Effect of a 50-Hz Electromagnetic Field at 500 μT on Parameters Related with the Cardiovascular System in Rats. Front Public Health. 2020 Apr 7;8:87.
- Zhou B, Tian R. Mitochondrial dysfunction in pathophysiology of heart failure. J Clin Invest. 2018 Aug 31;128(9):3716-3726.
- Zimmerman JJ, von Saint André-von Arnim A, McLaughlin J. Chapter 74 – Cellular Respiration, Editor(s): Bradley P. Fuhrman, Jerry J. Zimmerman. Pediatric Critical Care (Fourth Edition), Mosby, 2011, 1058-1072.